Scientists wielded giant lasers to simulate an exoplanet’s super-hot core
A molten core creates Earth's magnetic field, which protects life as we know it.
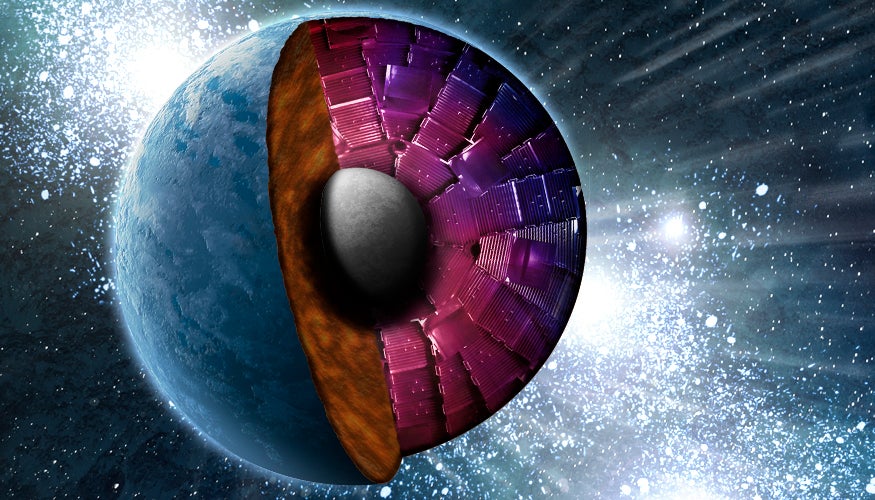
The molten cores of larger rocky exoplanets should stay hot longer than those within small worlds, according to a study published Thursday in the journal Science. That’s good news for interstellar explorers–because a molten core is probably required for life to develop on a planet.
Determining this feature of exoplanets required an experiment with giant lasers and an incredibly thin sliver of iron placed under unprecedented pressure. “We’re finding so many planets, and [one of] the big questions people have are: are these planets potentially habitable?” says Rick Kraus, a physicist at Lawrence Livermore National Laboratory who led the study.
To answer this question, researchers don’t normally start with thinking about a planet’s core. Instead, they ask whether the planet is the right distance from its star or whether it has water. But Kraus and his team wanted to find other ways to discern whether a planet is habitable.
They explored a planet’s ability to form a magnetosphere—a magnetic field that protects it from solar radiation, like the one around Earth does for us—as a window into habitability, Kraus says. Life as we know it wouldn’t be possible without the Earth’s magnetic field.
Magnetic fields are a result of molten planetary cores. Earth has a core composed mostly of iron, split into a solid inner core and a liquid outer core. Earth’s magnetic field is caused by the convection of the liquid iron, meaning how it swirls: The cooler, denser liquid areas sink to the bottom, while the hotter ones rise like wax in a lava lamp.
Studying an exoplanet’s core in a laboratory is difficult because there are few ways to recreate such intense pressures and temperatures. This is the first experiment to measure iron’s melting temperature at pressures exceeding those in Earth’s core, Kraus says. To achieve these extremes, the team needed some big lasers, specifically the National Ignition Facility in Lawrence Livermore National Lab–where big lasers are their specialty.
In the experiment, those lasers blasted a multilayered sample of iron. Layers of beryllium, a metal element, and some filters formed the outside of a super-thin iron “sandwich” while a piece of transparent lithium fluoride made up the other half, Kraus says. The outer beryllium layer heated up thousands of degrees in “a fraction of a billionth of a second,” he says, and that side of the sandwich cooked into a plasma. The plasma then expanded, driving an intense shockwave into the sample.
[Related: Saturn has a slushy core and rings that wiggle]
This process emulates the conditions that a section of hot iron would experience as it descends through a planet’s molten core. “You’re shocking the hell out of it,” says Peter Driscoll, geophysicist at Carnegie Science who models the core of Earth and other planets and was not involved in the study, who adds this was a difficult process to study. It destroys the sample, so the experimenters have to collect their data in one go. The process only produced “a couple of data points,” he says, but “these kinds of experiments are very valuable.”
As the sample reached peak pressures, another instrument tested whether that iron remained solid or liquid at key times, to help researchers home in on iron’s behavior at these high pressures and temperatures.
The team found that “as you increase the pressure, the temperature increases, quite rapidly,” Kraus says. For exoplanets, that means the larger they get, the longer it will take their cores to solidify. The super-Earths that are between four and six times Earth’s mass would take the longest, he says. The team estimates that it will take a total of 6 billion years for Earth’s core to solidify, whereas cores in large exoplanets of similar composition to Earth should take up to 30 percent longer.
“While that may sound sort of intuitive,” it wasn’t a given with all the different factors, Kraus says.
Measuring how iron melts in extreme conditions is so important because it tells you if and how a planet’s core will solidify, Driscoll says. Even at the boundary between Earth’s solid inner core and liquid outer core, scientists don’t know precisely what the temperature is–though it’s estimated to be near that of the sun’s surface, at roughly 10,000°F.
One issue with extrapolating these results to exoplanets is that those super-Earths can contain elements other than iron in their core, which would change their melting temperature by an unknown amount, Driscoll says. It will also be hard to predict how exoplanets cool because the mantle, the layer of hot rock surrounding the core, plays a huge role in how quickly the core can cool. And those exoplanet mantles could be made of “pretty much anything,” he says.
Venus, Driscoll says, is the go-to example of this disconnect. On paper its composition is very similar to Earth’s, but it lacks a magnetic field and plate tectonics.
Other factors can decide whether a magnetosphere will form, too, such as how well the material in the core lets heat or electricity flow through it. But these characteristics are difficult to measure, even on Earth–scientists have only succeeded in measuring the flow of heat through Earth’s core in the last decade. Still, Driscoll says, that would be, “the next thing to go after.”
Correction January 21, 2022: This article previously stated this experiment was the first to use iron at pressures exceeding Earth’s core. In fact, it was the first to measure iron’s melting point at those pressures.